|
Hi. Why can only Fe, Co and Ni be magnetic? This
question is common and on the web exist many
answers about this question. Most of these answers
pay attention to unpaired electrons of d shell. I
agree to pay attention to unpaired electrons, but
I believe there are other aspects that are as
important as unpaired electrons. In other words, I
think that unpaired electrons alone can't be
enough reason for this question. First of all it's
necessary to remember that the magnet is a
ferromagnetism material. Other aspects: Cr and Mn
have unpaired electrons more than Fe. So why can
't Cr and Mn be magnetic like Fe. We know Cr is
anti-fero-magnetic. In addition we know Mo isn't
anti-fero-magnetic like Cr. It is paramagnetic
while both of them have a number of unpaired
electrons. So in addition to unpaired electrons
there are others factors such that they are
effective in creating magnetism. What factors are
effective in creating magnetic properties? And how? |
Question Date: 2017-12-10 | | Answer 1:
Great question, Richard! Magnetism is
mysterious to most people because it can be
extremely complicated. But it has simple
origins. You’re right that unpaired d-shell
electrons play an important role. But that’s
effectively a single-atom view. The next level of
complexity is to look at nearest-neighbor
interactions.
What is the interaction between two atoms
sitting close to one another? How do the
intrinsic magnetic fields (spins) of the electrons
interact, and how much do the electrons themselves
overlap? The energy of that interaction is
called the Exchange Energy and it’s written:
E = -J * S1* S2,
where S1 and S2 are
the direction of the spins (intrinsic magnetic
field) of unpaired electrons on the first and
second atoms.
J is a coupling constant that can be
positive or negative , and its value depends
on an enormous number of factors including the
energy level and atomic shell the electrons
reside in (s, p, d, f), the crystal
structure and specific crystal site,
corrections due to Einstein’s theory of relativity
(for larger atoms like Mo), just to name a
few. For most materials, determining the value of
J requires supercomputers running quantum
mechanical simulations. I realize that is an
unsatisfying answer. There are teams of
researchers all over the world who specialize in
computing J, among other things, and much of the
time even they get an incorrect result.
Once J is known, we can say something
about what type of magnetic order we expect from
two atoms sitting next to each other, and then we
can extrapolate to large systems of atoms that
make up everyday objects like refrigerator
magnets. Magnetic systems always try to
minimize their energy . So if J is
negative, S1 will rotate around
until it is equal to -S2, so
that E is a negative number.
E = -(negative)(negative)(positive) =
(negative).
In other words, a material with negative J
will be anti-ferromagnetic. The spins will be
anti-parallel to one another, and since all
the internal magnetic fields cancel out, there
is no external magnetic field produced by
anti-ferromagnets. They won’t stick to your
fridge.
On the other hand, a positive value of J
will tend to cause S1 to rotate
to be parallel S2, so that E is
still minimized.
E = -(positive)(positive)(positive) =
(negative).
These materials have ferromagnetic ordering if
the value of J is high enough. Ferromagnets
produce an external field and some are strong
enough to stick to your fridge.
Thermal energy can easily wash out the
effects of the exchange energy if J is small.
That’s the difference between a paramagnet and and
a ferromagnet. A ferromagnet has a J high
enough to keep all the spins in a chunk of iron
(or whatever) pointed the same way, even though
temperature is always trying to randomize the
direction of the spins. A paramagnet has a
small J, but can be temporarily magnetized by
applying an external magnetic field (for example,
in a coil of wire with electrical current running
through) because paramagnets still have unpaired
electrons. With the assistance of an applied
field, some paramagnets would stick to your fridge
too. Most paramagnets become ferromagnets if you
cool them down enough and remove that randomizing
thermal energy.
That gives us enough to talk about Cr
and Mo . Cr is a small atom and is
stable in the bcc (body centered cubic) crystal
structure. The unpaired electrons are in the
3rd d-shell (3rd row of the periodic table). It
has a strong, negative exchange constant J, giving
antiferromagnetic order.
Mo is a larger atom but also crystallizes as
bcc. It has the same number of unpaired
electrons but they are in the 4th d-shell, further
out from the nucleus. That results in a very
different overlap between electrons on neighboring
atoms. For Mo, J ends up being small and positive,
giving paramagnetic order.
Also, as a side note, there are many more
magnetic materials than just the magnetic
elements. I work with the Heusler
compounds, which originally gained fame as the
first ferromagnetic compounds composed entirely of
non-magnetic elements. The first one was
Cu2MnAl (diamagnet, paramagnet,
paramagnet), which has stronger ferromagnetism
than Ni metal. In Heuslers, the
ferromagnetism (or anti ferromagnetism!) arises
because unpaired electrons actually get traded
between neighboring, dissimilar atoms. To do that
without violating the Pauli exclusion principle
(you can’t put two electrons with the same spi | | Answer 2:
Magnetism relies on the material having a net
magnetic moment, a quantity that determines
the amount of torque (how much it spins around)
the material experiences in a magnetic field. The
net magnetic moment for a bulk material, e.g. a
cubic inch of iron, requires the alignment, in one
direction, of small magnetic dipole moments.
Magnetic moment on a fundamental level exists
intrinsically (without any external stimulus) in
elementary particles, such as electrons and
particles that make up protons and neutrons,
because these particles have spin -- a form of
angular momentum. When these intrinsic magnetic
moments are not somehow balanced out, they can
align, resulting in a net magnetic dipole moment
in the bulk material, with or without the help of
an external field. What helps the alignment of
small magnetic moments into a large collective one
includes the number of electrons in a material,
how they pair, AND the crystalline structure and
microstructures. In fact, if we look closely at
iron, we see that for iron to become independently
magnetic, it needs to cool from high temperatures
to allow a change in domain structure, meaning
that the electron orientation of one domain (local
area) in the iron aligns with other domains so
that a net magnetic moment results.
The structural component of magnetism also
means that the material itself does not need to be
ferromagnetic. A really good example of this is
the compound CrO2, which is a
ferromagnetic but neither Cr nor O is
ferromagnetic alone. In short, alignment of small
magnetic moments is an essential part of magnetism
that cannot be entirely included in electron
pairing. The alignment can also help explain why
some metals don't respond to external magnetic
fields as readily as iron - the small magnetic
moments are not as easy to align because of
electron pairing, crystal structures, domains with
different orientations, and so on. | | Answer 3:
Great question! You are absolutely right, that
unpaired electrons in the d shell do not
explain why Fe, Co and Ni are ferromagnetic, Mn
and Cr are antiferromagnetic, and other transition
metals are nonmagnetic. If we imagine an isolated
atom that is not part of an extended solid (for
example, a single atom in a vacuum, or even a
molecule that contains a single magnetic atom in
it), then we only need to consider the presence
(or absence) of unpaired electrons to know whether
or not it is magnetic. Any atom with unpaired
electrons is magnetic. There are all sorts of
magnetic molecules that contain isolated metals
with unpaired electrons with all sorts of types of
atoms: even an oxygen molecule, O2, is
magnetic!
When you put a bunch of atoms together into an
extended metal, however, the physics changes a
lot. In metals, the valence electrons of each atom
join together to form a sea of electrons that is
shared across the whole metal. Electrons are no
longer associated with a single atom, and so the
concept of an individual atom's electron
configuration is meaningless for an extended
metal... we can't just count electrons and
orbitals to see if there are any unpaired
electrons like we can for an isolated atom! This
is why you have been unable to understand metallic
magnets using the concept of unpaired electrons.
The reason that some metals are magnetic while
others are not is actually very complicated—so
complicated that scientists are still trying to
fully understand this question! However, we do
understand some basic principles about magnetism
in metals.
For example:
1. Magnetism is more likely in the middle of a row
of the periodic table.
2. Magnetism is more likely at the top of a block
of the periodic table.
In the case of pure elemental metals, this
results in the only magnets being Fe, Co, Ni
(which are at the middle of the top of the
d-block) and Gd (which is at the middle of the top
of the f-block). However, there are lots of other
magnetic metals that are compounds with more than
one element in it. For example, the compound
MnCu2Al is a famous example of a ferromagnet. In
this compound, the Mn is actually the magnetic
element! In compounds like this, the above rules
still apply. There are lots of magnetic metallic
compounds based on Mn, Fe, Ni, and Co; some based
on V, Cr, Mo, Ru, Rh, Ni and Cu; and relatively
few based on other transition metals.
If you are interested in exploring some
metallic ferromagnetic compounds, you can check
out magnets.mrl.ucsb.edu, which contains
interactive applications for exploring data about
this type of compound.
Try, for example:
here
To see a plot showing different magnetic compounds
based on different transition metals.
Click Here to return to the search form.
|
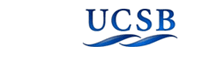 |
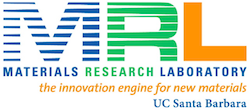 |
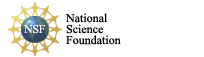 |
|
Copyright © 2020 The Regents of the University of California,
All Rights Reserved.
UCSB Terms of Use
|
|
|